Contact: rodolphe.boudot@femto-st.fr
Our group is involved in the study and development of compact and miniaturized vapor cell atomic clocks. These activities involve atomic spectroscopy, MEMS cell technologies, optics, analog and digital electronics and time and frequency metrology.
Our activities are divided into three main sections:
1/ Microwave CPT-based miniature atomic clocks
With the help of MEMS techniques, VCSEL lasers, integrated electronics and coherent population trapping (CPT) physics, we develop miniaturized atomic clocks that combine small volume (~15 cm3), low power consumption (150 mW), and fractional frequency stability at the level of 10-11 at 1 day integration. Such “pocket-size” atomic frequency references could replace quartz-crystal oscillators in numerous applications including network synchronization, satellite-based navigation systems or secure communications. These activities are performed in strong collaboration with FEMTO-ST MOSAIC Group [https://teams.femto-st.fr/MOSAIC/en] that brings strong expertise with MEMS cell technologies. Relevant efforts have been performed over the last years in collaboration with industrial partners.
2/ High-performance CPT-based Cs cell atomic clock
The goal is to demonstrate a CPT-based Cs cell atomic clock, with similar size and power consumption than commercially-available Cs beam clocks and a fractional frequency stability at the level of a few 10-13 t-1/2 and compatible with the level of 10-14 at 1 day integration time. Our laboratory-prototype clock combines an optimized CPT pumping scheme named push-pull optical pumping (proposed by Happer’s group in Princeton, in 2004) and a pulsed Ramsey-based interrogation protocol. Recently, the contribution of power-induced light-shifts to the frequency stability has been strongly rejected in the 10-16 range at 10 000 s with the implementation of a symmetric Auto-Balanced Ramsey interrogation protocol. The clock demonstrates a short-term stability of 2 10-13 t-1/2, with the potential to reach the level of 2.5 10-15 at 10 000 s, under quiet conditions.
3/ Microcell-based optical frequency references
We develop Cs microcell-based optical frequency references. These references are based on the excitation and detection of narrow atomic optical resonances in microfabricated cells. We have 3 in-progress projects. The first one uses dual-frequency sub-Doppler spectroscopy in a Cs cell at 895 nm. The second one uses the excitation of two-photon transition at 778 nm of Rb atom. The third one employs spectroscopy of the near-UV 6S1/2-7P1/2 transition of Cs atom at 459 nm.
1/ Miniaturized microwave CPT-based atomic clocks
Basics on CPT physics and CPT-based MAC architecture
Figure 1: CPT interaction in Cs atom represented by a 3- level Λ-scheme interacting with a bi-chromatic optical field. Both optical lines are frequency-split by 9.192 GHz (ground-state splitting), the CPT Cs clock transition. When the frequency difference between both optical lines exactly equals 9.192 GHz, atoms are optically-pumped in a coherent superposition of both ground states. In this so-called dark state, the transparency of the atomic vapor is increased, as shown on the right inset. The CPT resonance, with narrow line-width, can be used for the development of an atomic clock. In a CPT clock, the microwave interrogation signal is directly optically carried, preventing the use of a resonant cavity.
Basic blocks of a miniature atomic clock (MAC) are shown below on figure 2:
Figure 2: Schematic of CPT-based miniature Cs vapor cell atomic clock
The heart of a MAC is a micro-fabricated cell that contains alkali vapor, generally diluted with a pressure of buffer gas in order to slow down alkali atoms. Slight collisions between alkali atoms and buffer gas allow to increase the time for the atoms to reach the cell walls. This allows the detection of narrow CPT resonances (a few kHz line-width in mm-scale cell dimensions). The cell is typically heated at 80-90°C. A static magnetic field, parallel to the laser beam propagation direction, is applied to raise the Zeeman degeneracy. A magnetic shielding is used to prevent perturbations from the environment. Atoms in the cell interact with a laser beam coming from a VCSEL diode laser whose injection current is directly modulated at 4.596 GHz by a local oscillator to produce two first-order optical sidebands frequency-split by 9.192 GHz for CPT interaction. The laser beam is shaped, routed and polarized circularly with a quarter-wave plate. The light transmitted through the cell is detected by a photodiode. The output signal of the photodiode is used for various servo loops including in particular the laser frequency stabilization and the local oscillator frequency stabilization.
Cs vapor microcell technology
In 2007, we proposed and developed at FEMTO-ST an original pill-dispenser Cs vapor microcell technology. This cell consists of a DRIE-etched silicon substrate sandwiched between two anodically-bonded glass wafers. The cell contains two cavities. The first cavity contains a Cs pill dispenser. The second cavity is the cavity in which CPT interaction takes place. Both cavities are connected by channels. The originality of this cell technology is that Cs vapor is generated after complete sealing of the cell by local laser activation of the Cs pill dispenser. This cell technology is now industrially-transfered [36, 39].
Figure 3 shows a photograph of Cs microfabricated cells developed in FEMTO-ST.
Figure 3: Photographs of Cs vapor microfabricated cells. The Cs dispenser is visible in the square cavity. Courtesy from FEMTO-ST MOEMS Group.
Figure 4: Example of a CPT resonance detected in a Cs-Ne microfabricated cell.
In 2015, we reported an original Cs microcell architecture, named R-cell, designed for miniature atomic clocks. This cell combines diffraction gratings with anisotropically etched single-crystalline silicon sidewalls to route a normally-incident beam in a cavity oriented along the substrate plane.
Figure 5: Photograph of the R-cell architecture. Courtesy from FEMTO-ST MOEMS Group.
In 2017, we proposed and reported a method for filling alkali vapor cells with cesium from a dispensing paste, compatible with collective deposition processes. This filling method could reduce the cost and the complexity of miniature cells fabrication. Optical linear spectroscopy was performed over one year on such cells, showing a stable atomic density. One of these cells has been implemented in a lab-prototype CPT clock. A fractional frequency aging rate of about –4.4 10-12 per day has been measured, compliant with typical objectives of MACs.
In 2018, in collaboration with our industrial partner Tronics Microsystems, we have reported the characterization of Cs vapor microcells based on pill dispensers and fabricated in a MEMS foundry, according to a process compatible with mass-production [36]. More than three quarters of cells from 6-inch wafers are successfully filled with Cs vapor. Various cells of a given wafer have been characterized using CPT spectroscopy, demonstrating similar buffer gas (Ne) pressure with a standard deviation of about 2.5% and CPT resonances with similar line-width and contrast properties. In addition, frequency drifts mainly attributed to cell inner atmosphere variations have been investigated onto several cells over 250-500 hour measurements. The corresponding contribution at 1 day averaging time to the clock fractional frequency stability is estimated to be about 10-11 or lower. The fractional frequency stability of a clock prototype using such an industrial Cs-Ne microcell was measured to be 2.5 10-11 τ-1/2 up to 200 s averaging time, and better than 2 10-11 at 105 s. These performances tend to demonstrate that this vapor cell technology, compatible with mass-production, is suitable for industrial miniature quantum clocks or sensors.
In 2019, we reported a study on the mitigation of light-shift effects in a miniaturized atomic clock physics package, through the implementation of additional electronics stabilization loops [39]. The first loop stabilizes the actual temperature of the VCSEL chip using a compensation method. The second loop stabilizes the total microwave power absorbed by the laser to a value that maximizes the optical absorption and reduces the clock frequency dependence to laser-power variations.
In 2020, we reported the experimental implementation of an advanced light-shift mitigation interrogation protocol, applied to a continuous-wave microcell atomic clock. The interrogation sequence, named Auto-Compensated Shift (ACS), initially suggested by V. I. Yudin et al. [https://arxiv.org/pdf/1911.02935.pdf], is symmetrized to compensate for an atomic memory effect and imperfections of the laser power modulation pattern. We have demonstrated light-shift mitigation by a factor up to 170 by considering the non-linear dependence of the light-shift. The method allows a significant improvement of the clock Allan deviation after 1000 s. This approach could be applied to various kinds of atomic clocks, including Rb cell clocks or cell-based optical frequency references.
MEMS-based BAW-resonator oscillators
In 2014-2016, we reported the characterization of 2.3 GHz AlN-Sapphire high-overtone bulk acoustic resonators (HBARs), with a typical loaded Q-factor of 25 000, 15–20 dB insertion loss, and resonances separated by about 10 MHz. The temperature coefficient of frequency of these HBARs was measured to be about -25 ppm/K. A significant distortion of the HBAR resonance line-shape, attributed to non-linear effects, has been observed at high input microwave power. The power-induced fractional frequency variation of the HBAR resonance was measured. The residual phase noise of a HBAR was measured in the range of -110 to -130 dBrad2/Hz at 1Hz Fourier frequency, yielding an ultimate HBAR-limited oscillator Allan deviation of about 7 10-12 at 1 s integration time. A HBAR resonator was used for the development of a low phase noise 2.3 GHz oscillator, yielding an absolute phase noise of -60, -120, and -145 dBrad2/Hz for offset frequencies of 10 Hz, 1 kHz, and 10 kHz, respectively, in excellent agreement with the Leeson effect. Such a HBAR oscillator has been used as a local oscillator in a microcell CPT-based atomic clock.
Figure 6: Photograph of a AlN-Sapphire HBAR resonator (designed by CEA-LETI).
Figure 7: Phase noise performances of a 2.3 GHz HBAR oscillator (in red). Performances compared to those of a conventional MAC LO or to state-of-the-art ideally frequency-multiplied 100 MHz OCXO.
VCSELs
VCSEL laser sources combine a low threshold current, low power consumption and high-modulation bandwidth capabilities and are well-adapted to be used in miniature atomic clocks.
In the frame of the MAC-TFC project, in collaboration with Ulm University and LTF-UNINE, we reported the metrological characterization of custom-designed 894 nm VCSELs [12]. More recently, we reported the characterization of commercially-available 894 nm VCSELs [26].
Laboratory-prototype clocks
Figure 8 shows a microcell-based compact atomic clock physics package developed in the frame of the MAC-TFC project. Figure 9 shows this physics package embedded onto the pilot electronics card (developed by EPFL).
Figure 8: Picture of the MAC-TFC clock physics package.
Figure 9: Picture of the MAC-TFC clock. The physics package is connected to electronics to operate the clock. Electronics were developed by EPFL-IMT.
Recently, in the frame of the EFTS seminar, we have developed a MAC prototype for lab works and graduate students. This prototype is now used during EFTS seminars (http://efts.eu/dokuwiki/doku.php?id=current:01_program ).
Figure 10: Miniature clock prototype. Courtesy of J. Rutkowski and V. Maurice.
In 2019, we reported the operation of fully-miniaturized MAC physics package, with dimensions of 15*15*13 mm3. These physics package have been designed at FEMTO-ST and developed at Tronics Microsystems [39].
Ramsey-CPT spectroscopy in microfabricated cells.
In 2018, we reported the detection of Ramsey-CPT fringes detected in Cs-Ne microfabricated cells [32]. The properties of the central fringe clock transition were studied versus several experimental parameters, including the Ramsey sequence parameters, the input laser intensity and were compared to those of a standard CPT resonance (obtained in the CW mode). The hyperfince microwave coherence lifetime (T2) was measured in the range of 50-500 us, for different buffer gas pressure or cell temperatures. These studies could be of interest for the development of future Ramsey-CPT chip-scale atomic clocks.
Figure 11: Typical CPT resonance detected in the CW regime (black) and in the pulsed Ramsey-CPT regime (red), in a Cs-Ne MEMS cell.
In 2021, we have investigated the application of Ramsey spectroscopy for the development of a microcell atomic clock based on coherent population trapping (CPT). The sensitivity of the clock frequency to light-shift effects was studied. In comparison with the continuous-wave (CW) regime case, the sensitivity of the clock frequency to laser power variations is reduced by a factor up to 14 and 40.3 for dark times of 150 and 450 us, respectively, at the expense of an intensity 3.75 times higher for short-term stability optimization. We demonstrated that the Ramsey-CPT interrogation improves the clock Allan deviation for averaging times higher than 100 s. These results demonstrate that Ramsey-based interrogation protocols might be an attractive approach for the development of chip-scale atomic clocks with enhanced mid- and long-term stability [48].
In 2022, we demonstrated the implementation of symmetric auto-balanced Ramsey (SABR) interrogation in a microcell-based atomic clock for light-shift mitigation. A reduction of the clock frequency dependence to laser power, microwave power and laser frequency by more than two orders of magnitude, in comparison with the standard continuous-regime (CW) interrogation, was demonstrated (see ref [53] below).
2/ High-performance CPT-based Cs cell atomic clock
In 2011, in collaboration with LNE-SYRTE (S. Guérandel and E. de Clercq), we started the development of a high-performance CPT-based Cs cell atomic clock. Funded in the beginning by LNE, these activities have been pursued from 2013 to 2016 in the frame of a European project called MClocks, funded by EURAMET, and piloted by INRIM (coordinator: S. Micalizio). This project has been supported later by LabeX FIRST-TF.
Our high-performance CPT clock combines an optimized CPT pumping scheme, named push-pull optical pumping (PPOP), pioneerly proposed by Y. Y. Jau et al. in 2004 (Happer's group, Princeton, Phys. Rev. Lett. 93, 16, 2004) and a pulsed Ramsey-based interrogation protocol, yielding the detection of high-contrast Ramsey-CPT fringes. Our laboratory-prototype clock uses now a sophisticated Auto-Balanced Ramsey interrogation protocol, pioneerly proposed for optical clocks in PTB, Germany (see C. Sanner et al., Phys. Rev. Lett. 2018). This method allows the drastic reduction of light-shift effects. This CPT clock demonstrates to date a fractional frequency stability at the level of 2 10-13 τ-1/2 up to 10 000 s, reaching the level of 2.5 10-15 at 104 s. To our knowledge, these performances are the best performances ever reported for a CPT-based atomic clock.
Architecture of the CPT clock
Figure 12 shows the architecture of the Ramsey-CPT clock. It combines a DFB laser source tuned on the Cs D1 line, a fibered electro-optic modulator driven by a 4.596 GHz low noise microwave signal for generation of the CPT sidebands, an AOM for laser power stabilization and production of the Ramsey sequence and a Michelson delay-line and polarization orthogonalizer system for the generation of the PPOP scheme. The light is sent into a 5-cm long and 2-cm diameter buffer-gas filled Cs cell. The light at the output of the cell is detected by a photodiode. The output signal is exploited by a FPGA-based digital electronics board (developed by C. Calosso, INRIM) that generates the Ramsey sequence pattern and manages different servo loops. The local oscillator is an ultra-low phase noise 100 MHz OCXO frequency-multiplied to 4.596 GHz. The output signal of the 100 MHZ OCXO is at the end compared to a hydrogen maser for stability measurements.
Figure 12: Schematic of the Ramsey-CPT clock
Figure 13: Example of Ramsey-CPT fringes. The line-width of the central fringe equals 1/(2T), with T the free-evolution time in the Ramsey-CPT sequence.
Ultra-low noise microwave frequency synthesizers
In collaboration with INRIM, we developed in 2015 ultra-low phase noise microwave frequency synthesizers, dedicated to be used as local oscillators in high-performance Cs (or Rb) vapor cell atomic clocks [21]. These synthesizers exhibit extremely low phase noise and allow to reduce greatly the Dick effect contribution at the level of 3 10-14 at 1s, close to the shot noise limit.
I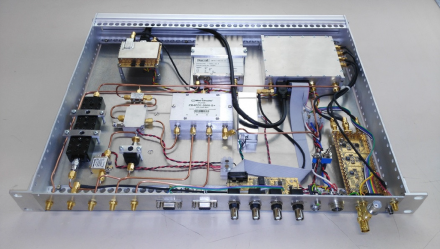
Figure 14: Photograph of a microwave frequency synthesizer developed by FEMTO-ST / INRIM [B. Francois, C. Calosso, R. Boudot].
Figure 15: Absolute phase noise performances of the microwave frequency synthesizer shown above. The pilot is a low noise 100 MHz OCXO (perfs in red at 100 MHz). The green data shows the absolute phase noise at 4.596 GHz. The green line is the phase noise at 4.596 GHz of the ideally frequency-multiplied 100 MHz OCXO.
Reduction of light-shift effects with Auto-Balanced Ramsey (ABR) interrogation protocol
Vapor-cell atomic clocks are widely appreciated for their excellent short-term fractional frequency stability and their compactness. However, they are known to suffer on medium and long time scales from significant frequency instabilities, generally attributed to light-induced frequency-shift effects.
In order to tackle this limitation, we investigated in 2018 the application of the Auto-Balanced Ramsey (ABR) interrogation protocol (see C. Sanner et al., Phys. Rev. Lett. 120, 053602 (2018)) onto our CPT-based pulsed hot-vapor Cs vapor-cell clock.
We have demonstrated that the ABR protocol, developed initially to probe the one-photon resonance of quantum optical clocks, can be successfully applied to a two-photon CPT resonance. The applied method, based on the alternation of two successive Ramsey-CPT sequences with unequal free-evolution times and the subsequent management of two interconnected phase and frequency servo loops, allows a relevant reduction of the clock-frequency sensitivity to light-shift effects [34].
This ABR-CPT protocol, improved further later with symmetrization (SABR-CPT), has allowed a drastic reduction of the clock frequency sensitivity to laser power variations, by a factor 80 in comparison with the standard Ramsey-CPT regime. This technique has reduced the contribution of the laser power-shift effect on the clock frequency stability to the level of a few 10-16 at 104 s [35].
Figure 16: Symmetric ABR-CPT sequence.
Figure 17 reports the Allan deviation of the clock in different conditions. The curve (a) shows the best performances ever obtained in the Ramsey-CPT regime. The curve (b) shows first results obtained in the ABR-CPT regime. The curve (c), extracted from a 5-days measurement, is obtained in the SABR-CPT regime, without compensation of the laser AM noise (explaining the degradation of the short-term stability). The curve (d), extracted from a 30 000 s data set with quiet experimental conditions, is obtained in the SABR-CPT regime, with additional compensation of the laser AM noise. In the last case, the clock demonstrates a clock fractional frequency stability of 2 10-13 τ-1/2, until almost 104 s, reaching the level of 2.5 10-15 at 104 s.
Figure 17: Allan deviation of the clock. (a) Ramsey-CPT, (b) First results obtained in the ABR-CPT regime, (c): SABR-CPT: extracted from a 5-days measurement, without compensation of the laser AM noise, (d): SABR-CPT, extracted from a 30 000 s data set with quiet experimental conditions, with additional compensation of the laser AM noise for improvement of the short-term stability. In the last case, the clock demonstrates a clock fractional frequency stability of 2 10-13 τ-1/2, until almost 104 s, reaching the level of 2.5 10-15 at 104 s.
In 2019, we contributed to the demonstration of variants of the ABR method, including Ramsey spectroscopy with Displaced Frequency Jumps [40] and the Combined Error Signal spectroscopy technique [37,41]. These two methods, demonstrated at NIST, are attractive alternatives for the mitigation of light-shift effects.
Annex studies: Wall coated Cs vapor cells.
In 2015, we reported the realization and characterization using coherent population trapping (CPT) spectroscopy of octa-decyl-trichlorosilane (OTS)-coated centimeter-scale Cs vapor cells. The dual-structure of the resonance line-shape, with presence of a narrow structure line at the top of a Doppler-broadened structure, was clearly observed. The line-width of the narrow resonance was compared to the line-width of an evacuated Cs cell and of a buffer gas Cs cell of similar size. The Cs-OTS adsorption energy was measured to be (0.42+/-0.03) eV, with a clock frequency shift rate of 2.710-9/K in fractional unit. A hyperfine population lifetime, T1, and a microwave coherence lifetime, T2, of 1.6 and 0.5 ms were reported, corresponding to about 37 and 12 useful bounces, respectively.
Figure 18: Photograph an OTS-coated cell.
Figure 19: CPT resonance in a Cs-OTS cell (compared to a pure Cs cell of similar dimensions).
3/ MEMS-cell optical frequency references
We have recently reported the detection of high-contrast sub-Doppler resonances in Cs MEMS vapor cells using a dual-frequency sub-Doppler spectroscopy (DFSDS) technique [24,31]. In this system, alkali thermal atoms confined in a mm-scale vapor cell interact with two orthogonally-polarized counter-propagating dual-frequency optical fields, yielding the detection of high-contrast sign-reversed sub-Doppler optical resonances. The observation of these enhanced-absorption spikes has been explained in an extended theoretical model (established in collaboration with D. Brazhnikov from Institute of Laser Physics, Novosibirsk, Russia) through the contribution of Zeeman dark states, hyperfine dark states and optical pumping effects [42].
Figure 20 shows the architecture of the MEMS cell based optical frequency reference.
Figure 20: Dual-frequency sub-Doppler spectroscopy setup.
Fgure 21 reports high-contrast sub-Doppler absorption spikes in a hot atomic vapor cell exposed to a dual-frequency laser field. Similar high-contrast resonances have been detected in mm-scale Cs vapor cells.
Figure 21: High-contrast sub-Doppler absorption spikes in a hot atomic vapor cell exposed to a dual-frequency laser field.
These high-Q factor (Q~5 10^7) optical resonances have been recently used for frequency stabilization of a diode laser, yielding a short-term frequency stability lower than 2 10-12 τ-1/2 until 10 s integration time. These promising performances are about 50-100 times better than those of commercial microwave CPT-based chip-scale atomic clocks and demonstrate the interest of this approach for the development of new-generation fully-miniaturized cell-based optical frequency references. Combined with integrated on-chip microresonator-based optical frequency combs used as optical-to-microwave frequency dividers, this clock architecture could constitute the basis for the generation of ultra pure microwave signals in a compact system with reasonable size and power consumption.
Publications
- D. Miletic, P. Dziuban, R. Boudot, M. Hasegawa, R. K. Chutani, G. Mileti, V. Giordano and C. Gorecki, Electron. Lett. 46, 15, 1069-1071 (2010).
- R. Boudot, P. Dziuban, M. Hasegawa, R. Chutani, S. Galliou, V. Giordano and C. Gorecki, Journ. Appl. Phys. 109, 014912 (2011).
- M. Hasegawa, R. K. Chutani, C. Gorecki, R. Boudot, P. Dziuban, V. Giordano, S. Clatot, J. Dziuban and L. Mauri, Sensors Actuators A - Phys. 167, 594-601 (2011).
- R. Boudot, D. Miletic, P. Dziuban, P. Knapkiewicz, J. Dziuban, C. Affolderbach, G. Mileti, V. Giordano and C. Gorecki, Optics Express 19, 4, 3106-3114 (2011).
- R. Boudot, M. D. Li, V. Giordano, N. Rolland, P. A. Rolland, P. Vincent, Rev. Sci. Instr. 82, 034706 (2011).
- O. Kozlova, R. Boudot, S. Guerandel and E. De Clercq, IEEE Trans. Instrum. Meas. 60, 7, 2262-2266 (2011).
- D. Miletic, C. Affolderbach, M. Hasegawa, R. Boudot, C. Gorecki and G. Mileti, Appl. Phys. B: Lasers Opt. 109, 89–97 (2012). DOI 10.1007/s00340-012-5121-7
- X. Liu and R. Boudot, IEEE Trans. Instr. Meas. 61, 10, 2852-2855 (2012). http://dx.doi.org/10.1109/TUFFC.2012.2493
- R. Boudot, X. Liu, P. Abbé, R. K. Chutani, N. Passilly, S. Galliou, C. Gorecki and V. Giordano, IEEE Trans. Ultrason. Ferroelec. Freq. Contr. 59, 11, 2584-2587 (2012). http://dx.doi.org/10.1109/TUFFC.2012.2502
- R. Boudot and E. Rubiola, IEEE Trans. Ultrason. Ferroelec. Freq. Contr. 59, 12, 2613-2624 (2012).
- X. Liu, J. M. Merolla, S. Guérandel, C. Gorecki, E. De Clercq and R. Boudot, Phys. Rev. A 87, 013416 (2013). https://doi.org/10.1103/PhysRevA.87.013416
- F. Gruet, E. Kroemer, L. Bimboes, D. Miletic, C. Affolderbach, A. Al-Samaneh, D. Wahl, R. Boudot, G. Mileti and R. Michalzik, Optics Express 21, 5, 5781-5792 (2013). https://doi.org/10.1364/OE.21.005781
- X. Liu, J.M. Merolla, S. Guérandel, E. De Clercq and R. Boudot, Optics Express 21, 10, 12451-12459 (2013). https://doi.org/10.1364/OE.21.012451
- M. Hasegawa, R. K. Chutani, R. Boudot, L. Mauri, C. Gorecki, X. Liu and N. Passilly, J. Micromech. Microeng. 23, 055022 (2013). doi:10.1088/0960-1317/23/5/055022
- R. Salut, C. Gesset, G. Martin, B. Assouar, P. Bergonzo, R. Boudot, O. Elmazria and S. Ballandras, Elsevier, Microelectron. Eng. 112, 133-138 (2013).
- J. M. Friedt, R. Boudot, G. Martin and S. Ballandras, Rev. Sci. Instr. 85, 094704 (2014). http://dx.doi.org/10.1063/1.4894264
- B. François, J-M. Danet, C. E. Calosso and R. Boudot, Rev. Sci. Instr. 85, 094709 (2014). http://dx.doi.org/10.1063/1.4896043
- M. Abdel Hafiz, V. Maurice, R. Chutani, N. Passilly, C. Gorecki, S. Guérandel, E. De Clercq and R. Boudot, Journ. Appl. Phys. 117, 184901 (2015). http://dx.doi.org/10.1063/1.4919841
- E. Kroemer, M. Abdel Hafiz, V. Maurice, B. Fouilland, C. Gorecki and R. Boudot, Opt. Express 23, 14, 18373-18380 (2015). https://doi.org/10.1364/OE.23.018373
- R. K. Chutani, V. Maurice, N. Passilly, C. Gorecki, R. Boudot, M. Abdel Hafiz, P. Abbé, S. Galliou, J. Y. Rauch and E. De Clercq, Nature Sci. Rep. 5, 14001 (2015). DOI: 10.1038/srep14001
- B. Francois, C. E. Calosso, M. Abdel Hafiz, S. Micalizio and R. Boudot, Rev. Sci. Instr. 86, 094707 (2015). http://dx.doi.org/10.1063/1.4929384
- M. Abdel Hafiz and R. Boudot, Journ. Appl. Phys. 118, 124903 (2015). http://dx.doi.org/10.1063/1.4931768
- T. Daugey, J. M. Friedt, G. Martin and R. Boudot, Rev. Sci. Instr. 86, 114703 (2015). http://dx.doi.org/10.1063/1.4935172
- M. Abdel Hafiz, G. Coget, E. De Clercq and R. Boudot, Opt. Lett. 41, 13, 2982-2985 (2016). http://dx.doi.org/10.1364/OL.41.002982
- M. Abdel Hafiz, X. Liu, S. Guérandel, E. De Clercq and R. Boudot, Journal of Physics: Conference Series, 723 (1), 012013 (2016).
- E. Kroemer, J. Rutkowski, V. Maurice, R. Vicarini, M. Abdel Hafiz, C. Gorecki and R. Boudot, Applied Optics 55, 31, 8839-8847 (2016). http://dx.doi.org/10.1364/AO.55.008839
- R. Boudot, G. Martin, J. M. Friedt and E. Rubiola, Journal of Applied Physics 120, 224903 (2016). http://dx.doi.org/10.1063/1.4972102
- P. Yun, F. Tricot, C. E. Calosso, S. Micalizio, B. Francois, R. Boudot, S. Guérandel and E. de Clercq, Phys. Rev. Applied 7, 014018 (2017). DOI: 10.1103/PhysRevApplied.7.014018
- M. Abdel Hafiz, G. Coget, P. Yun, S. Guérandel, E. De Clercq and R. Boudot, Journal of Applied Physics 121, 104903 (2017). http://dx.doi.org/10.1063/1.4977955
- V. Maurice, J. Rutkowski, E. Kroemer, S. Bargiel, N. Passilly, R. Boudot, C. Gorecki, L. Mauri and M. Moraja, Appl. Phys. Lett. 110, 164103 (2017). http://dx.doi.org/10.1063/1.4981772
- M. Abdel Hafiz, D. Brazhnikov, G. Coget, A. Taichenachev, V. I. Yudin, E. De Clercq and R. Boudot, New Journal of Physics 19, 073028 (2017). https://doi.org/10.1088/1367-2630/aa7258
- R. Boudot, V. Maurice, C. Gorecki and E. de Clercq, Journal of Optical Society America B 35, 5, 1004-1010 (2018). https://doi.org/10.1364/JOSAB.35.001004
- D. Brazhnikov, G. Coget, M. Abdel Hafiz, V. Maurice, C. Gorecki and R .Boudot, IEEE Transactions Ultrasonics Ferroelectrics Frequency Control 65, 6, 962-972 (2018). https://doi.org/10.1109/TUFFC.2018.2811319
- M. Abdel Hafiz, G. Coget, M. Petersen, C. Rocher, S. Guérandel, T. Zanon-Willette, E. de Clercq and R. Boudot, Physical Review Applied 9, 064002 (2018). https://doi.org/10.1103/PhysRevApplied.9.064002
- M. Abdel Hafiz, G. Coget, M. Petersen, C. Calosso, S. Guérandel, E. de Clercq and R. Boudot, Applied Physics Letters 112, 244102 (2018). https://doi.org/10.1063/1.5030009
- R. Vicarini, V. Maurice, M. Abdel Hafz, J. Rutkowski, C. Gorecki, N. Passilly, L. Ribetto, V. Gaff, V. Volant, S. Galliou and R. Boudot, Sensors Actuators, Phys. A 280, 99-106 (2018). https://doi.org/10.1016/j.sna.2018.07.032
- V. I. Yudin, A. Taichenachev, M. Yu Basalaev, T. Zanon-Willette, T. E. Mehlstauber, R. Boudot, J. W. Pollock, M. Shuker, E. A. Donley and J. Kitching, New Journal of Physics 20, 123016 (2018). https://doi.org/10.1088/1367-2630/aaf47c
- D. Brazhnikov, M. Petersen, G. Coget, V. Maurice, N. Passilly, C. Gorecki and R. Boudot, Phys. Rev. A 99, 062508 (2019). https://doi.org/10.1103/PhysRevA.99.062508
R. Vicarini, M. Abdel Hafiz, V. Maurice, N. Passilly, E. Kroemer, L. Ribetto, V. Gaff, C. Gorecki, S. Galliou and R. Boudot, Mitigation of temperature-induced light-shift effects in miniaturized atomic clocks, IEEE Transactions on Ultrasonics Ferroelectrics and Frequency Control 66, 12, 1962-1967 (2019). https://doi.org/10.1109/TUFFC.2019.2933051
M. Shuker, J. W. Pollock, R. Boudot, V. I. Yudin, A. V. Taichenachev, J. Kitching and E. A. Donley, Physical Review Letters 122, 113601 (2019). https://doi.org/10.1103/PhysRevLett.122.113601
M. Shuker , J. W. Pollock, R. Boudot, V. I. Yudin, A. V. Taichenachev, J. Kitching and E. A. Donley, Applied Physics Letters 114, 141106 (2019). https://doi.org/10.1063/1.5093921
D. Brazhnikov, S. Ignatovich, V. Vishnyakov, R. Boudot, and M. Skvortsov, Optics Express 27, 25, 36034-36045 (2019). https://doi.org/10.1364/OE.27.036034
- J. P. McGilligan, K. R. Moore, A. Dellis, G. D. Martinez, E. de Clercq, P. F. Griffin, A. S. Arnold, E. Riis, R. Boudot and J. Kitching, Applied Physics Letters 117, 054001 (2020). https://doi.org/10.1063/5.0014658
- V. I. Yudin, M. Yu Basalaev, A. V. Taichenachev, J. W. Pollock, Z. L. Newman, M. Shuker, A. Hansen, M. T. Hummon, R. Boudot, E. A. Donley and J. Kitching, General methods for suppressing the light shift in atomic clocks using power modulation, Physical Review Applied 14, 024001 (2020). https://doi.org/10.1103/PhysRevApplied.14.024001
- M. Abdel Hafiz, R. Vicarini, N. Passilly, C. E. Calosso, V. Maurice, J. W. Pollock, A. V. Taichenachev, V. I. Yudin, J. Kitching and R. Boudot, Protocol for light-shift compensation in a continuous-wave microcell atomic clock, Physical Review Applied 14, 034015 (2020). https://doi.org/10.1103/PhysRevApplied.14.034015
- R. Boudot, J. P. McGilligan, K. R. Moore, V. Maurice, G. D. Martinez, A. Hansen, E. de Clercq and J. Kitching. Enhanced observation time of magneto-optical traps using micro-machined non-evaporable getter pumps. Sci Rep 10, 16590 (2020). https://doi.org/10.1038/s41598-020-73605-z
- D.V. Brazhnikov, S.M. Ignatovich, I.S. Mesenzova, A.M. Mikhailov, M.N. Skvortsov, A.N. Goncharov, V.M. Entin, I.I. Ryabtsev, R. Boudot, E. Taskova, E. Alipieva, C. Andreeva, S. Gateva, “Nonlinear enhanced-absorption resonances in compact alkali-vapor cells for applications in quantum metrology”, J. Phys. Conf. Ser., vol. 1859, 012019 (2021).DOI: 10.1088/1742-6596/1859/1/012019
- C. Carlé, M. Petersen, N. Passilly, M. Abdel Hafiz, E. de Clercq and R. Boudot, Exploring the use of Ramsey-CPT spectroscopy for a microcell-based atomic clock, IEEE Transactions on Ultrasonics Ferroelectrics and Frequency Control 68, 10, 3249-3256 (2021). https://ieeexplore.ieee.org/document/9445113
- A. Gusching, M. Petersen, N. Passilly, M. Abdel Hafiz, E. de Clercq and R. Boudot, Short-term stability of Cs microcell-stabilized lasers using dual-frequency sub-Doppler spectroscopy, J. Opt. Soc. Am. B 38, 10 (2021). https://doi.org/10.1364/JOSAB.438111
- A. Bregazzi, P. Griffin, A. S. Arnold, D. P. Burt, G. Martinez, R. Boudot, J. Kitching, E. Riis and J. P. McGilligan, A simple imaging solution for chip-scale laser cooling, Applied Physics Letters 119, 184002 (2021). https://doi.org/10.1063/5.0068725
- R. Boudot, M. Abdel Hafiz, M. Petersen, C. E. Calosso and E. Rubiola, All-optical microwave feedback oscillator with atomic cell resonator, Applied Physics Letters 120, 044101 (2022). https://doi.org/10.1063/5.0072799
A. M. Mikhailov,R. Boudotand D. Brazhnikov, Line shape of the sub-Doppler resonance in alkali-metal atomic vapors in the field of counterpropagating bichromatic laser beams, Journal of Experimental and Theoretical Physics (JETP) 133, 6, 696-710 (2021).
- M. Abdel Hafiz, C. Carlé, N. Passilly, J. M. Danet, C. E. Calosso and R. Boudot, Light-shift mitigation in a microcell-based atomic clock with Symmetric Auto-Balanced Ramsey spectroscopy, Appl. Phys. Lett. 120, 064101 (2022). https://doi.org/10.1063/5.0082156
M. Petersen, M. Abdel Hafiz, E. de Clercq and R. Boudot, Microwave phase detection of coherent population trapping resonance in Cs vapor cell, J. Opt. Soc. Am. B 39, 3, 910-916 (2022).https://doi.org/10.1364/JOSAB.446828
- D. Teyssieux, R. Boudot, C. Fluhr and J. Millo, Phase noise mitigation of the microwave-to-photonic conversion process using feedback on the laser current, Journal of Optical Society America B, 39, 11, 3108-3113 (2022). https://doi.org/10.1364/JOSAB.475377
- V. Maurice, C. Carlé, S. Keshavarzi, R. Chutani, S. Queste, L. Gauthier-Manuel, JM. Cote, R. Vicarini, M. Abdel Hafiz, R. Boudot and N. Passilly, Wafer-level vapor cells filled with laser-actuated hermetic seals for integrated atomic devices, Nature Microsystems and Nanoengineering 8, 129 (2022). https://doi.org/10.1038/s41378-022-00468-x
- P. Yun, R. Boudot and E. de Clercq, Coherent population trapping with high common-mode noise rejection using differential detection of simultaneous dark and bright resonances, Physical Review Applied 19, 024012 (2023). https://doi.org/10.1103/PhysRevApplied.19.024012
- A. Gusching, J. Millo, I. Ryger, R. Vicarini, M. Abdel Hafiz, N. Passilly, and R. Boudot, Cs microcell optical reference with frequency stability in the low 10-13 range at 1 s, Optics Letters 48, 6, 1526-1529 (2023). https://doi.org/10.1364/OL.485548
- C. Carlé, M. Abdel Hafiz, S. Keshavarzi, R. Vicarini, N. Passilly and R. Boudot, Pulsed-CPT Cs-Ne microcell atomic clock with frequency stability below 2x10^-12 at 10^5 s, Optics Express 31, 5, 8160 (2023). https://doi.org/10.1364/OE.483039
- C. Carlé, S. Keshavarzi, A. Mursa, P. Karvinen, R. Chutani, S. Bargiel, S. Queste, R. Vicarini, P. Abbé, M. Abdel Hafiz, V. Maurice, R. Boudot and N. Passilly, Reduction of helium permeation in microfabricated cells using alumino-silicate substrates and Al2O3 coatings, Journal of Applied Physics 133, 214501 (2023). https://doi.org/10.1063/5.0151899
- D. Teyssieux, J. Millo, E. Rubiola and R. Boudot, Phase noise of a microwave photonic channel: direct-current versus external electro-optic modulation, Journal of Optical Society America B 41, 2, 442-446 (2024). https://doi.org/10.1364/JOSAB.514025
- C. M. Rivera-Aguilar, M. Callejo, A. Mursa, R. Vicarini, M. Abdel Hafiz, N. Passilly and R. Boudot, Operation of a Ramsey-CPT microcell atomic clock with driving current-based power modulation of a vertical-cavity surface-emitting laser, Applied Physics Letters 124, 114102 (2024). https://doi.org/10.1063/5.0196975
- E. Klinger, A. Mursa, C. M. Rivera-Aguilar, R. Vicarini, N. Passilly and R. Boudot, Sub-Doppler spectroscopy of the Cs atom 6S1/2 - 7P1/2 transition at 459 nm in a microfabricated vapor cell, Optics Letters 49, 8, 1953-1956 (2024). https://doi.org/10.1364/OL.514866
- C. Carlé, A. Mursa, P. Karvinen, S. Keshavarzi, M. Abdel Hafiz, V. Maurice, R. Boudot and N. Passilly, On the reduction of gas permeation through the glass windows of micromachined vapor cells using Al2O3 coatings, Journal of Applied Physics 136, 085102 (2024). https://doi.org/10.1063/5.0213432